Covid-19 Breakthrough Symptoms
While a vaccine can save you from a deadly infection, it won't always prevent mild sickness if you contract coronavirus.
Even if you are among those who experience a breakthrough infection, vaccinated individuals are much less likely to have severe symptoms when it comes to respiratory issues, fevers, aches or gastrointestinal pains. It's why it can be confusing for most vaccinated individuals to differentiate a potential cold or stomach bug from a breakthrough COVID-19 infection — symptoms are usually much milder than for those who are unvaccinated.
Whether you've been fully vaccinated for a few months' time or are still building immunity after your first shot, keeping the following symptoms in mind can help you determine if it's worth seeking out a COVID-19 test or immediate medical attention.
Vaccinated individuals may experience any of the following symptoms during a breakthrough COVID-19 infection:
- Fever and widespread chills across the body
- Nasal congestion, runny nose and sore throat
- Coughing
- Labored breathing, chest pain and respiratory congestion
- Partial or full loss of taste and smell
- Fatigue and joint pain
- Diarrhea, nausea and vomiting
- Vertigo and eye inflammation, although rarer among patients
- Headaches, and 'brain fog,' even into recovery periods
- Difficulty multitasking or trouble focusing on a task at hand
You may experience a certain pairing of symptoms at the same time, or develop a certain side effect first before experiencing another — or, in asymptomatic cases, you may experience no symptoms at all. A breakthrough case is consistent with other outbreaks that have occurred in 2020 and beyond, and treatment remains largely the same as well, Dr. Sullivan adds.
https://www.goodhousekeeping.com/health/a37182862/breakthrough-covid-symptoms-cdc/
What are the parts of a coronavirus?
Coronaviruses are a large family of viruses, some of which infect humans.
The coronavirus at the root of COVID-19 is the newest known member of this family. And like other coronaviruses that infect people, the new coronavirus causes respiratory disease, among other symptoms.
At their core, coronaviruses contain a genetic blueprint called RNA (beige), similar to DNA. The single-stranded RNA acts as a molecular message that enables production of proteins needed for other elements of the virus.
Bound to this string of RNA are nucleoproteins— (dark blue discs)—proteins that help give the virus its structure and enable it to replicate.
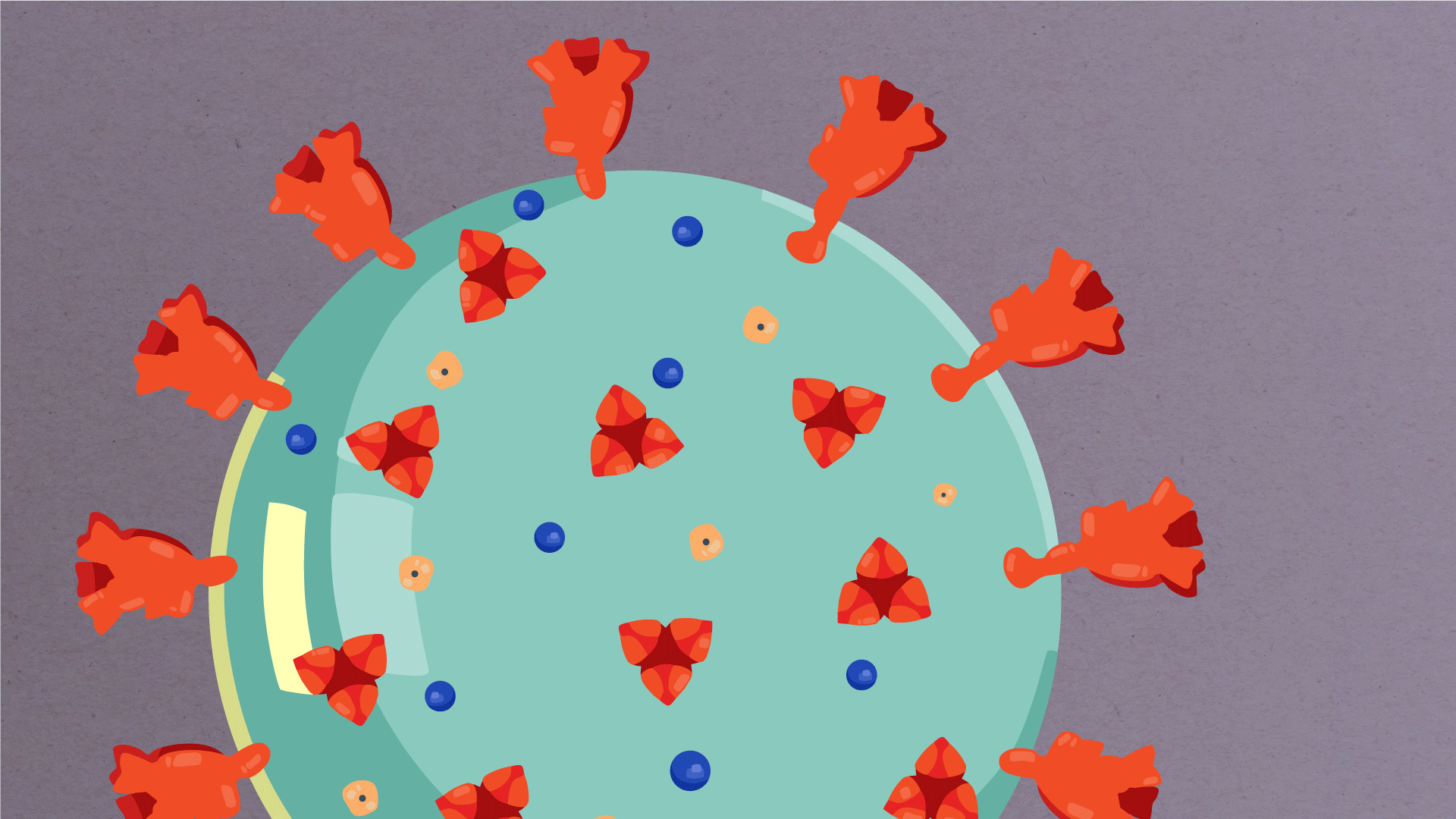
Encapsulating the RNA genome is the viral envelope (teal), which protects the virus when it is outside of a host cell. This outer envelope is made from a layer of lipids, a waxy barrier containing fat molecules. As well as protecting the precious genetic cargo, this layer anchors the different structural proteins needed by the virus to infect cells. Envelope proteins (dark blue dots) embedded in this layer aid the assembly of new virus particles once it has infected a cell.
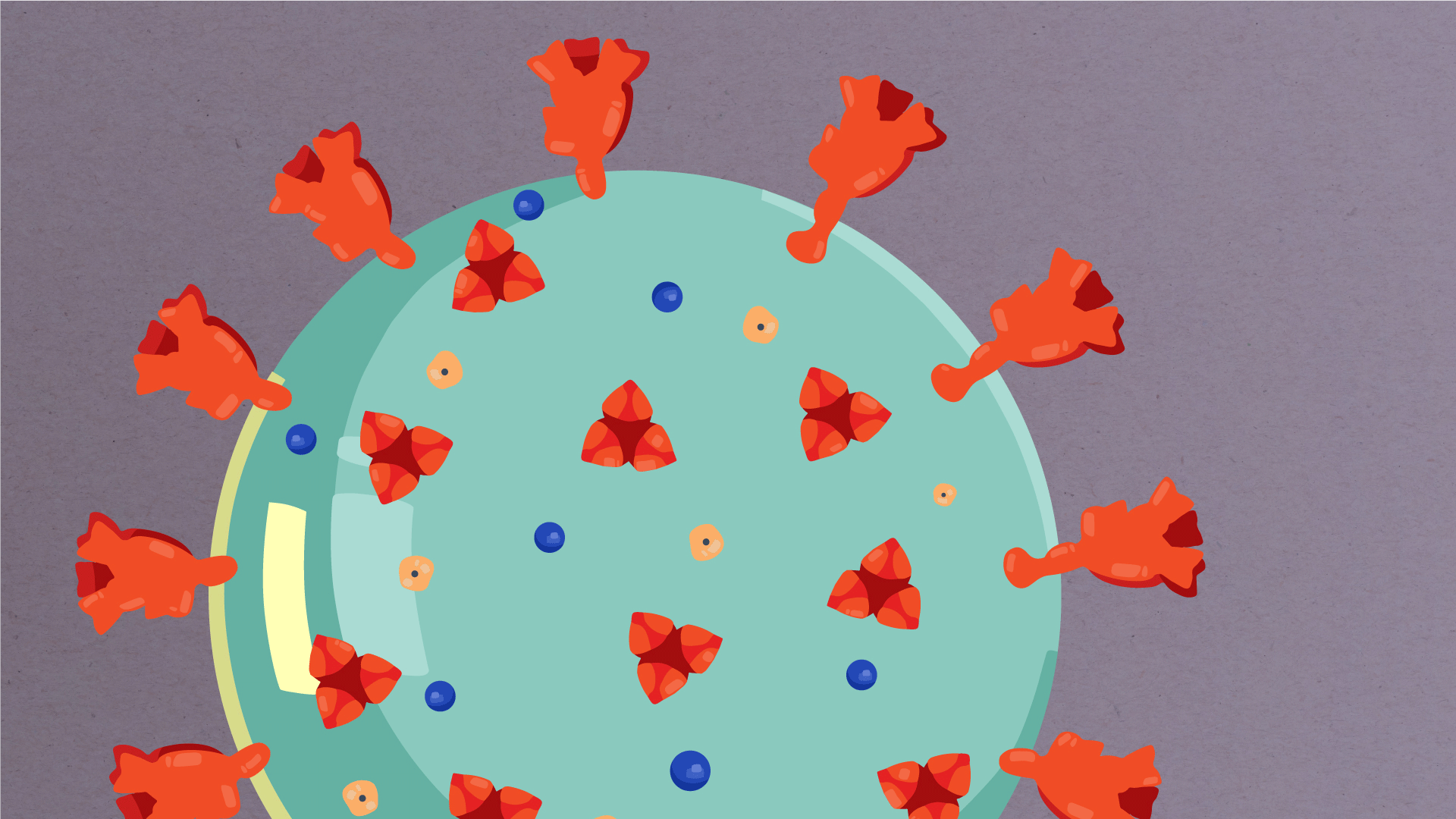
The bulbous projections seen on the outside of the coronavirus are spike proteins (red-orange). This fringe of proteins gives the virus its crown-like appearance under the microscope, from which the Latin name corona is derived. The spike proteins act as grappling hooks that allow the virus to latch onto host cells and crack them open for infection. Like all viruses, coronaviruses are unable to thrive and reproduce outside of a living host.
https://www.scripps.edu/covid-19/science-simplified/parts-of-a-coronavirus/
The Weird Way Coronaviruses Assemble Their Offspring
First, the virus enters an animal’s body through a mucus-lined surface — the nose, mouth or eyes. It uses its crown of proteins to fuse to a host cell surface or a cellular package called an endosome, which is engulfed by cells.
Once inside the host cell, the virus uses the first two-thirds of its genome to replicate. It copies its genome and makes structural proteins critical to form new viruses that will soon escape the host cell. But instead of going straight to the cell membrane to get ready to be shipped out of the host cell, the virus stops at a pancakelike structure called the Golgi complex, a kind of post office for the cell that sorts and processes proteins and spits them out of the cell after enclosing the proteins in a compartment called a vesicle.
At the Golgi complex, coronaviruses assemble new viruses and package them, using a piece of the Golgi complex’s membrane to form the new virus’s lipid envelope. Then, the Golgi complex stuffs the newly formed viruses into vesicles, which make their way to the cell surface.
“It’s not the most efficient way to assemble viruses and get them out of a cell,” says Machamer. “But there has to be a reason why this mechanism has persisted.”
Other viruses also use the Golgi complex to assemble their offspring. The most well known one is rubella (German measles).
Other viruses, such as West Nile, hepatitis C and Zika, use a different cellular structure to assemble new viruses: endoplasmic reticulum, which is made of membranes that foster protein production in the cell.
Machamer recently found that coronaviruses neutralize the pH of the Golgi complex, potentially paving a better path to help the germs, with their spiky halo, escape cells.
https://www.hopkinsmedicine.org/news/articles/the-weird-way-coronaviruses-assemble-their-offspring
How do viruses enter cells, replicate and head for the exits?
For a virus to spread, it must first find a way into a cell. But, said Carette, “penetrating a cell’s perimeter isn’t easy.” The outer membranes of cells are normally tough to get into without some kind of special pass. Viruses have ways of tricking cells into letting them in, though.
Typically, a portion of the virus’s cloak will have a strong affinity to bind with one or another protein that dots the surfaces of one or another cell type. The binding of the virus with that cell-surface protein serves as an admission ticket, easing the virus’s invasion of the cell.
The viral genome, like ours, is an instruction kit for the production of proteins the organism needs. This genome can be made up of either DNA, as is the case with all creatures except for certain viruses, or DNA’s close chemical relative RNA, which is much more flexible and somewhat less stable. SARS-CoV-2’s genome is made of RNA, as are the genomes of most mammal-infecting viruses.
In addition to the gene coding for its capsid protein, every virus needs another gene for its own version of an enzyme known as a polymerase. Inside the cell, viral polymerases generate numerous copies of the invader’s genes, from whose instructions the cell’s obedient molecular assembly line produces capsid subunits and other viral proteins.
Among these can be proteins capable of co-opting the cellular machinery to help viruses replicate and escape, or of tweaking the virus’s own genome — or ours. Depending on the type of virus, the genome can contain as few as two genes — one for the protein from which the capsid is built, the other for the polymerase — or as many as hundreds.
Capsids self-assemble from their subunits, often with help from proteins originally made by the cell for other purposes, but co-opted by the virus. Fresh copies of the viral genome are packaged inside newly made capsids for export.
Often, the virus’s plentiful progeny punish the good deed of the cell that produced them by lysing it — punching holes in its outer membrane, busting out of it and destroying the cell in the process.
But enveloped viruses can escape by an alternative process called budding, whereby they wrap themselves in a piece of membrane from the infected cell and diffuse through the cell’s outer membrane without structurally damaging it. Even then, the cell, having birthed myriad baby viruses, is often left fatally weakened…
The virus breaks into a cell
Assistant professor of chemical engineering and subcellular-compartment spelunker Monther Abu-Remaileh, PhD, described two key ways the coronavirus breaks into a cell and seeks comfort there, and how it might be possible to bar one of those entry routes with the right kind of drug.
Here’s one way: Once the coronavirus locks on to a cell, its greasy envelope comes into contact with the cell’s equally greasy outer membrane. Grease loves grease. The viral envelope and cell membrane fuse, and the viral contents dump into the cell.
The other way is more complicated. The viral attachment can set off a process in which the area on the cell’s outer membrane nearest the spot where the contact has been made caves in — with the virus (happily) trapped inside — until it gets completely pinched off, forming an inbound, membrane-coated, liquid-centered capsule called an endosome inside the cell. (To visualize this, imagine yourself with a wad of bubble gum in your mouth, blowing an internal bubble by inhaling, and then swallowing it. In this analogy, you’re the cell and all your skin, beginning with your lips, constitutes the cell’s outer membrane.)
Enclosed in this endosome is the viral particle that set the process in motion. The little devil has just hooked itself a ride into the cell’s inner sanctum. At this point, the viral particle consists of its envelope, its capsid and its enclosed genome — a blueprint for the more than two dozen proteins the virus needs and the invaded cell doesn’t provide.
But the endosome doesn’t remain an endosome indefinitely, Abu-Remaileh told me. Its mission is to become another entity called a lysosome, or to fuse with an existing lysosome.
Lysosomes serve as cells’ recycling factories, breaking down large biomolecules into their constituent building blocks for reuse. For this, they need an acidic environment, generated by protein pumps on their surface membranes that force protons into these vesicles.
The building internal acidity activates enzymes that chew up the cloistered coronavirus’s spike proteins. That brings the virus’s envelope in contact with the vesicle membrane and enables their fusion.
The viral genome gets squirted out into the greater expanse of the cell. There, the viral genome will find and commandeer the raw materials and molecular machinery required to carry out its genetic instructions. That machinery will furiously crank out viral proteins — including the customized polymerase SARS-CoV-2 needs to replicate its own genome. Copies of the genome and the virus’s capsid proteins will be brought together and repackaged into viral progeny…
How the coronavirus reproduces
SARS-CoV-2 has entered the cell, either by fusion or by riding in like a Lilliputian aquanaut, stealthily stowed inside an endosome. If things go right for the virus, it fuses with the endosome’s membrane and spills its genome out into the (relatively) vast surrounding cellular ocean.
That lonely single strand of RNA that is the virus’s genome has a big job to do — two, in fact, Judith Frydman, PhD, professor of biology and genetics, told me — in order to bootstrap itself into parenting a pack of progeny. It must replicate itself in entirety and in bulk, with each copy constituting the potential seed of a new viral particle. And it must generate multiple partial copies of itself — sawed-off sections that serve as instructions, telling the cell’s protein-making machines, called ribosomes, how to manufacture the virus’s more than two dozen proteins.
To do both things, the virus needs a special kind of polymerase. Every living cell, including each of ours, uses polymerases to copy its DNA-based genome and to transcribe its contents (the genes) into RNA-based instructions that ribosomes can read.
The SARS-CoV-2 genome, unlike ours, is made of RNA, so it’s already ribosome-friendly, but replicating itself means making RNA copies of RNA. Our cells never need to do this, and they lack polymerases that can.
SARS-CoV-2’s genome, though, does carry a gene coding for an RNA-to-RNA polymerase. If that lone RNA strand can find and insert itself into a ribosome, the latter can translate the viral polymerase’s genetic blueprint into a working protein. Fortunately for the virus, there can be as many as 10 million ribosomes in a single cell.
Once made, the viral polymerase cranks out not only multiple copies of the full-length viral genome — replication — but also individual viral genes or groups of them. These snippets can clamber aboard ribosomes and command them to produce the entire repertoire of all the proteins needed to assemble numerous new viral offspring.
These newly created proteins include, notably, more polymerase molecules. Each copy of the SARS-CoV-2 genome can be fed repeatedly through prolific polymerase molecules, generating myriad faithful reproductions of the initial strand.
Well, mostly faithful. We all make mistakes, and the viral polymerase is no exception; actually it’s pretty sloppy as polymerases go — much more so than our own cells’ polymerases, Carette and Frydman told me. So the copies of the initial strand — and their copies — are at risk of being riddled with copying errors, aka mutations.
However, coronavirus polymerases, including SARS-CoV-2’s, come uniquely equipped with a sidekick “proofreader protein” that catches most of those errors. It chops out the wrongly inserted chemical component and gives the polymerase another, generally successful, stab at inserting the proper chemical unit into the growing RNA sequence…
The final round in the cellular boxing ring
In addition to replicating its full-length genome, the virus has to make lots of proteins. And it knows just how. Those RNA snippets spun off by the viral polymerase are tailored to play by the cell’s protein-making rules — well, up to a point. They fit into ribosomes exactly as do the cell’s own strands of “messenger RNA” copied from the cell’s genes by its own DNA-reading polymerases. So-called mRNAs are instructions for making proteins.
But there’s a hitch: Among the proteins the virus forces ribosomes to manufacture are some that, once produced, bite the hand that fed them. Certain newly made viral proteins home to ribosomes in the act of reading one or another of the cell’s mRNA strands, hook themselves onto the strand and stick stubbornly, stalling out the ribosome until the cell’s mRNA strand falls apart.
The genomic RNA strands the virus generates, though, all have little blockades on their front ends that protect them from being snagged on the cell’s ribosomes by the viral wrecking crew. The result: The cell’s protein-making assembly line is overwhelmingly diverted to the production of viral proteins. That’s a two-fer: It both increases viral-component production and stifles the infected cell’s natural first line of defense…
How the coronavirus destroys cells / How scientists attempt to disarm it
https://stanmed.stanford.edu/2020issue2/how-coronavirus-destroys-cells-treatments.html
Understanding How COVID-19 Vaccines Work
What You Need to Know
- COVID-19 vaccines are safe and effective.
- You may have side effects after vaccination, but these are normal.
- It typically takes two weeks after you are fully vaccinated for the body to build protection (immunity) against the virus that causes COVID-19.
- If you are not vaccinated, find a vaccine. Keep taking all precautions until you are fully vaccinated.
-
If you are fully vaccinated, you can resume activities that you did prior to the pandemic. Learn more about what you can do when you have been fully vaccinated.
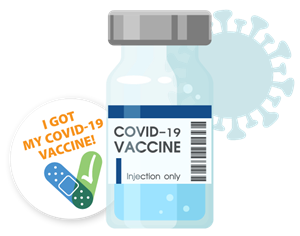
The Immune System—the Body’s Defense Against Infection
To understand how COVID-19 vaccines work, it helps to first look at how our bodies fight illness. When germs, such as the virus that causes COVID-19, invade our bodies, they attack and multiply. This invasion, called an infection, is what causes illness. Our immune system uses several tools to fight infection. Blood contains red cells, which carry oxygen to tissues and organs, and white or immune cells, which fight infection. Different types of white blood cells fight infection in different ways:
- Macrophages are white blood cells that swallow up and digest germs and dead or dying cells. The macrophages leave behind parts of the invading germs, called “antigens”. The body identifies antigens as dangerous and stimulates antibodies to attack them.
- B-lymphocytes are defensive white blood cells. They produce antibodies that attack the pieces of the virus left behind by the macrophages.
- T-lymphocytes are another type of defensive white blood cell. They attack cells in the body that have already been infected.
The first time a person is infected with the virus that causes COVID-19, it can take several days or weeks for their body to make and use all the germ-fighting tools needed to get over the infection. After the infection, the person’s immune system remembers what it learned about how to protect the body against that disease.
The body keeps a few T-lymphocytes, called “memory cells,” that go into action quickly if the body encounters the same virus again. When the familiar antigens are detected, B-lymphocytes produce antibodies to attack them. Experts are still learning how long these memory cells protect a person against the virus that causes COVID-19.
How COVID-19 Vaccines Work
COVID-19 vaccines help our bodies develop immunity to the virus that causes COVID-19 without us having to get the illness.
Different types of vaccines work in different ways to offer protection. But with all types of vaccines, the body is left with a supply of “memory” T-lymphocytes as well as B-lymphocytes that will remember how to fight that virus in the future.
It typically takes a few weeks after vaccination for the body to produce T-lymphocytes and B-lymphocytes. Therefore, it is possible that a person could be infected with the virus that causes COVID-19 just before or just after vaccination and then get sick because the vaccine did not have enough time to provide protection.
Sometimes after vaccination, the process of building immunity can cause symptoms, such as fever. These symptoms are normal and are signs that the body is building immunity.
Learn more about getting your vaccine.
Types of Vaccines
Currently, there are three main types of COVID-19 vaccines that are authorized and recommended or undergoing large-scale (Phase 3) clinical trials in the United States.
Below is a description of how each type of vaccine prompts our bodies to recognize and protect us from the virus that causes COVID-19. None of these vaccines can give you COVID-19.
- mRNA vaccines contain material from the virus that causes COVID-19 that gives our cells instructions for how to make a harmless protein that is unique to the virus. After our cells make copies of the protein, they destroy the genetic material from the vaccine. Our bodies recognize that the protein should not be there and build T-lymphocytes and B-lymphocytes that will remember how to fight the virus that causes COVID-19 if we are infected in the future.
- Protein subunit vaccines include harmless pieces (proteins) of the virus that causes COVID-19 instead of the entire germ. Once vaccinated, our bodies recognize that the protein should not be there and build T-lymphocytes and antibodies that will remember how to fight the virus that causes COVID-19 if we are infected in the future.
- Vector vaccines contain a modified version of a different virus than the one that causes COVID-19. Inside the shell of the modified virus, there is material from the virus that causes COVID-19. This is called a “viral vector.” Once the viral vector is inside our cells, the genetic material gives cells instructions to make a protein that is unique to the virus that causes COVID-19. Using these instructions, our cells make copies of the protein. This prompts our bodies to build T-lymphocytes and B-lymphocytes that will remember how to fight that virus if we are infected in the future.
Some COVID-19 Vaccines Require More Than One Shot
To be fully vaccinated, you will need two shots of some COVID-19 vaccines.
- Two shots: If you get a COVID-19 vaccine that requires two shots, you are considered fully vaccinated two weeks after your second shot. Pfizer-BioNTech and Moderna COVID-19 vaccines require two shots.
- One Shot: If you get a COVID-19 vaccine that requires one shot, you are considered fully vaccinated two weeks after your shot. Johnson & Johnson’s Janssen COVID-19 vaccine only requires one shot.
If it has been less than two weeks since your shot, or if you still need to get your second shot, you are NOT fully protected. Keep taking steps to protect yourself and others until you are fully vaccinated (two weeks after your final shot).
https://www.cdc.gov/coronavirus/2019-ncov/vaccines/different-vaccines/how-they-work.html
How COVID-19 vaccine works, potential side effects | Coronavirus |
https://health.ucdavis.edu/coronavirus/covid-19-vaccine/how-covid-19-vaccines-work.html
What Is Coronavirus? | Johns Hopkins Medicine
https://www.hopkinsmedicine.org/health/conditions-and-diseases/coronavirus
Coronavirus disease (COVID-19): How is it transmitted?
https://www.who.int/news-room/q-a-detail/coronavirus-disease-covid-19-how-is-it-transmitted
Typical Ingredients in the Pfizer Vaccine
https://www.tiktok.com/@alexdainis/video/6902543055639956741
https://www.tiktok.com/@laughterinlight/video/6972942775264349445
Summary: Pathophysiology of Covid-19 with Dr. Mike
Test Exercises: Test 1; With all that above understood, all parts of this technical video below should make complete sense now.
Print this image and tape it to your body somewhere
Hospitals once again running out of ICU beds and ECMO machines are good reasons to share this terrific mRNA vaccine explanation by @ScientistSwanda pic.twitter.com/Gk4b76BaZZ
— Avraham Z. Cooper, MD (@AvrahamCooperMD) August 8, 2021
What's the Difference?
B-cells and T-cells

When the body is invaded by bacteria, a virus or parasites, an immune alarm goes off, setting off a chain reaction of cellular activity in the immune system. Macrophages or other innate immune cells, such as basophils, dendritic cells or neutrophils, may be deployed to help attack the invading pathogen. Those cells often do the job, and the invader is destroyed. But sometimes, when the body needs a more sophisticated attack, it turns to its T-cells and B-cells. These cells are the special ops of the immune system—a line of defense that uses past behaviors and interactions to learn to recognize specific foreign threats and attack them when they reappear.
They may also play a critical role in the development and treatment of cancer. T-cells, especially, are the focal point for two emerging immunotherapy treatments: checkpoint inhibitors, which have been federally approved to treat multiple cancers, and CAR T-cell therapy, which is being studied in clinical trials as a potential treatment for cancers of the bloodstream, such as leukemia and lymphoma.
How does the immune system work?
The immune system is made up of two armies of cells: innate and acquired. Innate immune cells are the body's first line of defense. They quickly respond to foreign cells to fight infection, battle a virus or defend the body against bacteria. Our acquired immunity—also called adaptive immunity—uses T-cells and B-cells when invading organisms slip through that first line. These cells take longer to develop, because their behaviors evolve from learned experiences, but they tend to live longer than innate cells. Adaptive immune cells remember foreign invaders after their first encounter and fight them off the next time they enter the body. This is the fundamental premise for how vaccines work—using a small, harmless amount of protein from a disease to allow the immune system to recognize that protein if the pathogen were to invade the body.
B-cells and T-cells are also called lymphocytes. “There are primary and secondary lymphoid organs involved in the complex development of lymphocytes,” says Pamela Crilley, DO, Chair of the Department of Medical Oncology at Cancer Treatment Centers of America® (CTCA). “The primary lymphoid tissues in the initial generation of B- and T-lymphocytes are the bone marrow and the thymus.”
B-cells fight bacteria and viruses by making Y-shaped proteins called antibodies, which are specific to each pathogen and are able to lock onto the surface of an invading cell and mark it for destruction by other immune cells. B-lymphocytes and cancer have what may be described as a love-hate relationship. For example, B-cells sometimes inhibit tumor development by producing antibodies that may attack cancer cells or oncogenic viruses, such as human papillomavirus (HPV), which is responsible for most cervical, anal, penile and other reproductive cancers. Other times, regulatory B-cells may release immune-suppressive cytokines that stifle an anti-tumor response. Also, B-cells are far more likely than T-cells to mutate into a liquid cancer such as chronic lymphocytic leukemia (CLL) or B-cell lymphoma.
What Do T-Cells Do?
There are two main types of T-cells: helper T-cells and killer T-cells. Helper T-cells stimulate B-cells to make antibodies and help killer cells develop. Killer T-cells directly kill cells that have already been infected by a foreign invader. T-cells also use cytokines as messenger molecules to send chemical instructions to the rest of the immune system to ramp up its response. Activating T-cells against cancer cells is the basis behind checkpoint inhibitors, a relatively new class of immunotherapy drugs that have recently been federally approved to treat lung cancer, melanoma and other difficult cancers. Cancer cells often evade patrolling T-cells by sending signals that make them seem harmless. Checkpoint inhibitors disrupt those signals and prompt the T-cells to attack the cancer cells.
Researchers are also developing a technology called CART therapy, in which T-cells are engineered to attack specific cancer cells. In this potential treatment, which is still in clinical trials, a patient’s T-cells are collected and genetically engineered to produce chimeric antigen receptors (CAR). This is designed to allow the T-cells to recognize a specific protein on the tumor cells. These engineered CAR T-cells are grown by the billions in the laboratory and then infused into a patient’s body, where the cells are designed to multiply and recognize the cancer cells that express the specific protein. This technology, also called adoptive cell transfer, is generating excitement among researchers as a potential next-generation immunotherapy treatment.